Extracellular Vesicles and the Promise of Continuous Liquid Biopsies
Article information
Abstract
The rapid and accurate diagnosis of patients with minimally invasive procedures was once only found in science fiction. However, the discovery of extracellular vesicles (EVs) and their near ubiquity in body fluids, coupled with the advent of inexpensive next generation sequencing techniques and EV purification protocols, promises to make science fiction a reality. Purifying and sequencing the RNA content of EV from routine blood draws and urine samples are likely to enable pathologists and physicians to diagnose and track the progress of diseases in many inaccessible tissues in the near future. Here we present the evolutionary background of EV, summarize the biology of EV formation and cargo selection, and discuss the current barriers to making continuous liquid biopsies through the use of EV a science reality.
The production and release of membrane-bound vesicles by cells (which we will refer to generally as extracellular vesicles [EVs] in this review) is a process found in members of all three domains, including Archaea [1,2], Bacteria [3-5], and Eukaryotes (Fig. 1) [3-15]. EVs contain a diverse complement of proteins, nucleotides, and lipids [16,17], perform a large variety of functions, including antigen processing [18], host-parasite communication [19] and competition with other species [1], and are hypothesized to be formed by multiple mechanisms [8]. The observation of EVs in fluids such as saliva [20], urine [21], and blood [22] as well as the visualization and selection of EVs released from many human cells and cell lines including placenta (syncytiotrophoblasts [23], HTR-8, and JEG-3 [24]), kidneys [25], and blood cells (including lymphocytes [14], platelets [26], and reticulocytes [27]) argues for the continued function of EV in humans when coupled with the history of convergent evolution and conservation of function of EVs. In this review, we will first present some of the current hypotheses for the functions of human-derived EV, and second suggest how the phenomenon of EV production can be utilized for the remote sensing of inaccessible tissues and discuss the roadblocks to immediate utilization.
PRODUCTION AND FUNCTION OF EXTRACELLULAR VESICLES
EVs have been found in almost every human body fluid,28 including urine [21,29], blood [22,30], saliva [20,31], cerebrospinal fluid (CSF) [32], synovial fluid [33], semen [34], breast milk [35], amniotic fluid [36], aqueous humor (from cadavers) [37], lymph [38], and bronchoalveolar lavage fluid [39]. Because of the ubiquity of EVs, the mechanism of production and the likely functions of EVs have been the subject of intense study.
Production of extracellular vesicles
Eukaryotes generate multiple kinds of EVs. The major classes include exosomes, which are generated from multi-vesicular endosomes (MVEs), shedding microvesicles, which originate from the plasma membrane, apoptotic blebs, which result from programmed cell death, and gesicles, which are produced by vesicular stomatitis virus and potentially incorporate specific cargo proteins [40]. Exosomes are generated by the inward budding of MVEs and early endosomes which is mediated by the actions of endosomal sorting complexes required for transport (ESCRTs), ceramides, and tetraspanins [41]. The inward budding results in the potentially selective incorporation of cytoplasmic components including RNA, DNA, and proteins into the lumen of the exosome, as well as membrane lipids and transmembrane proteins. If the resultant MVEs are targeted to the plasma membrane instead of the lysosome, the fusion of MVEs with the plasma membrane leads to the release of exosomes. The fusion event is mediated by the action of multiple Rab proteins, including RAB27A (RAB27A, member RAS oncogene family), RAB35 (RAB35, member RAS oncogene family), and RAB11A (RAB11A, member RAS oncogene family) [42] in addition to the action of the cytoskeleton and fusion machinery (soluble NSF attachment protein receptors [SNAREs], etc.) [41]. The proteins involved in the processing of MVEs into EVs, including the ESCRTs, Rabs, and SNAREs, are present across the eukaryotes, which argues for EV production in at least the last common eukaryotic ancestor. The presence of orthologs of ESCRTs in archaea suggests that the last universal common ancestor may also have produced EVs [43]. However, since these proteins are also essential to ubiquitination pathways, it is possible that the conservation of EV production is a by-product of the conservation of the targeting of proteins to the lysosome for degradation.
The budding of microvesicles from the plasma membrane requires the actin-myosin machinery and small GTPases such as ADP ribosylation factor 6 (ARF6), but does not appear to require ESCRTs, although there is evidence that ESCRT-I is associated with some microvesicles. Further advances in microscopy, including autofluorescence contrast microscopy, are likely to enable the visualization of EV production [44].
Cargo selection in extracellular vesicles
The content of EVs often differs significantly from the cellular compartments from which the EVs are generated, both in terms of membrane composition and surface markers, as well as the contents of the lumen (RNA, DNA, and proteins) (Fig. 2) [16,17]. While some of the differences in membrane composition may be due purely to free energy considerations within the membrane such as curvature and charge, the enrichment of specific RNA motifs (ACCAGCCU, CAGUGAGC, and UAAUCCCA) [45] and protein surface markers (epithelial cell adhesion molecule [EPCAM], Erb-b2 receptor tyrosine kinase 2 [ERBB2], protein tyrosine phosphatase, receptor type C [PTPRC], or CD63 molecule [CD63]) [46] argues for the selective packaging of EV contents. Proteins that may fulfill the role of RNA sorting include members of the ESCRT-II complex as well as the RNA-induced silencing complex (RISC) (including trinucleotide repeat containing 6A [TNRC6A] and argonaute 2 [AGO2, RISC catalytic component] [41,47]), but the complete picture of the RNA sorting is still unclear. For example, it is not yet clear whether the sorting of RNA into exosomes is for the primary purpose of export in EV or if it is a side effect of targeting RNA to the lysosome which is sometimes exploited to provide export in EV. Pulse-chase experiments with labelled RNA may help elucidate the kinetics of this transport, and thereby determine whether specific RNAs are targeted for secretion, or if the process is RNA agnostic.
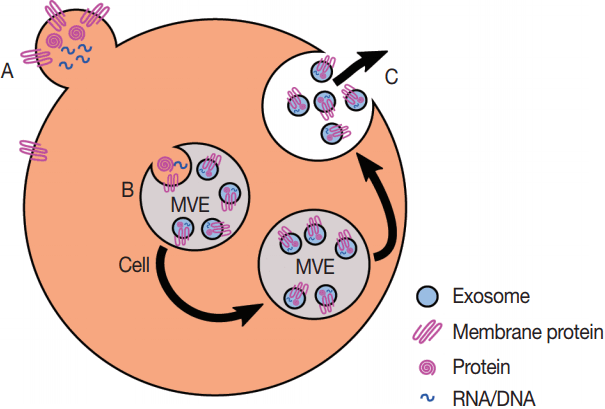
Production of exosomes and microvesicles in cells. Microvesicles are generated from the budding of the plasma membrane (A). Exosomes are generated from the inward budding of multi-vesicular endosomes (MVEs) by the action of multiple proteins (B) and result in the release of exosomes (C) if the MVE is targeted to the plasma membrane instead of the late endosome.
Function of extracellular vesicles
There are multiple hypotheses for the ancestral function of EVs. The first set of hypotheses deal with the function of the content of EVs; the second with the need to enclose the content of EVs within vesicles, and the degree of importance of the vesicle membrane surface markers. EVs from gram-negative bacteria have been shown to contain Quorum sensing molecules [48], toxins (Shiga toxin [49], ClyA [50]), and immune system modulators [5,8]. Enteroparasites such as nematodes have also been shown to modulate innate immunity by transferring small RNAs (miR-100 and Y RNA) to mammalian cells [19,51].
In humans, EVs can modulate the immune system by presenting antigens to antigen presenting cells (APCs) through internalization or fusion, being released by APCs to activate natural killer (NK), CD8+ T, and CD4+ T cells, directly activating neutrophils, macrophages and NK cells, repressing immune responses through CD8+ T cells, NK cells, or myeloid derived suppressor cells, and finally by conveying opsonins and complement components to modulate phagocytosis and apoptotic cell phagocytosis [52]. These mechanisms are even exploited by some cancer cells by releasing EVs which induce apoptosis of activated T cells [52]. During human pregnancy, EVs with a distinct population of mRNA and miRNA are released from villous trophoblasts in the placenta; these EVs also contain FasL and TRAIL which promote apoptosis and subsequent immunosuppression of T cells [53,54]. These EVs are likely utilized by the placenta to help maintain allograft tolerance during pregnancy [24].
Using vesicles for packaging EVs can reduce the exposure of the contents to host proteases and antibodies [48], as well as coupling cargo with targeting receptors/agonists without requiring direct targeting molecule to cargo interactions. This mechanism of packaging hints at the vast potential of therapeutic cargo inside of EVs with specific surface markers for the treatment of many diseases, such as cancer, where a cargo needs to be delivered to a precise tissue or cell type which is otherwise difficult to access (Table 1) [28].
EXTRACELLULAR VESICLES FOR DIAGNOSIS AND THERAPEUTICS
EVs in diagnosis
Diagnosing cancer using EVs
The contents of EVs have been found to be modified in multiple different cancers, including colorectal cancer [77], prostate cancer [78,79], glioblastomas [61,80], and breast cancer [81], as well as many others (see Maas et al. [82] for review). Multiple patents have already been filed to use the contents of EVs for diagnosis (see Urbanelli et al. [28] for review) so it is likely only a matter of time before EVs are used in the clinic for diagnosis.
Remote sensor of inaccessible organs
The most promising use of EVs is as a remote sensor of organs which are inaccessible to routine monitoring. In patients with breast cancer, EVs isolated from the serum contain higher levels of glypican 1 (GPC1); in patients with early pancreatic cancer, in addition to elevated GPC1, EVs contained mutant transcripts of KRAS (KRAS proto-oncogene, GTPase) [83]. EVs released by the placenta during pregnancies and identified in maternal blood (and potentially other body fluids, such as urine) will enable clinicians to routinely monitor the health of placentation and pregnancy throughout gestation without invasive tests [24,53,54,84]. There is also mounting evidence that EVs package and may spread misfolded proteins associated with neurodegenerative diseases, which can be detected in CSF and blood, including the scrapie isoform of cellular prion protein (PrPsc) that is involved in Creutzfeldt-Jakob disease [85]. EVs may potentially be useful in the diagnosis and progression tracking of similar neurodegenerative diseases.
Typing of infections which are otherwise inaccessible
Because the releasing of EVs is widely conserved in all three domains of life, it is possible that EVs which are present in accessible fluids, such as urine, saliva, or blood, may be of bacterial or fungal origins. Sequencing RNA present in EVs may identify the infectious agent responsible even though the source tissue is diffcult to access, enabling more effective treatment.
Additional potential uses of EVs
EVs which are introduced from an exogenous source have the potential to be used as the therapeutic agent, in much the same way as liposomes have been utilized [28,86]. Because EVs can be generated using simple bioreactors using appropriate engineered cell lines coupled with immunoaffnity or other purification [87], EVs based therapeutics may prove to be more specific, uniform, and cheaper to produce than current liposome based technology. In theory, any transmembrane receptor or receptor agonist can be coupled with any other cargo which is small enough to fit into an EV and injected, potentially targeting widespread metastases or locations which are otherwise inaccessible to targeted therapeutics [28,86].
ROADBLOCKS TO FURTHER USE OF EXTRACELLULAR VESICLES IN DIAGNOSIS
There are three distinct ways in which isolated EVs can be used in diagnosis. The first is a marker-only approach, where the concentration of the marker(s) in EV in the fluid of interest predicts disease or phenotype, and no other disease or healthy condition is likely to result in elevated concentrations of the marker(s). The second is a decomposition-based multi-marker approach, where the relative contribution of each tissue to the sub-sample of EVs is estimated using tissue-specific markers, and is correlated with differences in disease- or phenotype-relevant markers in that subsample. The third is a single-EV multi-marker approach, where single EV are interrogated to identify their tissue of origin and disease- or phenotype-relevant marker concentrations.
EV isolation
The methods of isolation currently used include ultracentrifugation, density gradient centrifugation, size exclusion through membranes, polymeric precipitation, immunoaffinity capture, and microfluidics [46,88,89]. Ultracentrifugation is an easily executed protocol, but also results in the enrichment of proteins with high sedimentation rates which are not bound to exosomes, including major vault protein, heparan sulfate proteoglycan 2 (HSPG2), fatty acid synthase (FASN), and the 26S proteasome [88]. Density gradient centrifugation reduces the contamination with high sedimentation rate protein complexes, but requires additional western blotting steps to verify that the density fraction isolated contains exosomes and has lower effciency than immunoaffinity capture.
Immunoaffinity capture using bead-conjugated antibodies to EPCAM or another exosome marker (such as ERBB2, PTPRC, or CD63) is simpler than density gradient centrifugation, and produces exosomes of high purity, but is limited to exosomal populations which express specific surface markers and may potentially miss novel exosome populations. Therefore, discovery experiments looking for novel populations of exosomes should use density gradient centrifugation, whereas established assays looking at known exosome populations can use appropriate immunoaffinity capture methods. Witwer et al. [89] and future recommendations of the International Society for Extracellular Vesicles provide valuable recommendations for investigators examining EVs in humans.
Normalization of EV content across tissues/samples
For circulating miRNA, Fesler et al. [90] suggests uniform volumes of plasma or exogenous sequences and points out that endogenous control genes may vary between subjects (as well as in the control population).
Identification of tissue of origin
Because EVs located in body fluids can potentially originate from any source tissue for which there is a path to that fluid, accurate diagnostics of remote tissues also require identification of the tissue of origin of a particular EV or set of EVs. As the miRNA and mRNA contents of EVs came from cells in the originating tissue, miRNA and mRNA which are specific to those originating tissue but found within EVs in body fluid indicate that at least some of the EVs came from the original tissue. For example, the presence of high abundance placenta-specific transcripts such as LGALS14 in a sample of EVs would indicate that at least some of the EVs came from the placenta. Determining the fraction of EVs which are from a particular tissue of origin would allow for changes in miRNA and mRNA abundance over time to be assigned to a particular source tissue when multiple samples of different per-tissue abundances are obtained at a single timepoint. Marker-free (such as CellCODE [91]) and/or marker-dependent methods will likely be necessary to determine the proportion of exosomes which come from different source tissues in a mixed population.
Quantification of a single EV
In cases where the source tissue has not been assayed, an alternative is the (much more diffcult) process of sequencing individual EV separately. Presumably, the advances in single-cell and single nucleus sequencing will provide the tools for single EV sequencing as a side benefit. Previous work by Smith et al. [92] using laser tweezers Raman spectroscopy that identified the lipid content of EVs on a per-EV basis identified multiple exosome subtypes which do not correlate strongly with the originating cell type, and therefore may represent different exosome subclasses which may be present in a single bulk exosome preparation. However, Raman spectroscopy is very slow and does not easily enable identification of the contents of exosomes. In an alternative approach, Kibria et al. [93] used micro flow cytometry after differential centrifugation to analyze CD44 (CD44 molecule) surface marker intensity on individual exosomes. This overcomes the limitations of typical flow cytometers, which can only detect minimum particle sizes of 200–500 nm, which is much larger than typical EVs. We suspect that the combination of micro flow cytometry with droplet based barcoding and sequencing techniques can enable the sequencing of the RNA contents of individual EVs in the not-too-distant future.
CONCLUSION
EVs are poised to fulfill the promise of routine liquid biopsies in healthcare, whether used for diagnosing disease, tracking the effectiveness of therapy, or providing insight into multiple diseases as well as potentially tracking the progress of pregnancies in a non-invasive fashion.
Notes
Conflicts of Interest
No potential conflict of interest relevant to this article was reported.
Acknowledgements
We would like to thank the insightful comments of Priyadarshini Pantham and Monica Uddin on drafts of this manuscript. This work was funded in part by the National Institutes of Health 1R21ES027878-01 grant to DEW.